Contact
Address
Universität Erlangen-Nürnberg
Chair of Computer Science 5 (Pattern Recognition)
Martensstrasse 3 91058 Erlangen Germany
Powered by
|
Dr.-Ing. Alexander Brost
Alumnus of the Pattern Recognition Lab of the Friedrich-Alexander-Universität Erlangen-Nürnberg
Providing physicians all required information.
IPCAI 2011, LNCS, Vol. 6689, 2011, pp. 133-144
Introduction
Atrial fibrillation (AFib), which is the most common arrhythmia, leads to an increased risk of stroke for the patient. After the first treatment approaches using radio-frequency ablations, this method has now become an accepted treatment option. Catheter ablation procedures are performed in electrophysiology (EP) labs usually equipped with modern C-arm X-ray systems. These devices often provide 3-D imaging of the heart to overcome the issue that soft-tissue of the heard is difficult to see in X-ray images. Electro-anatomic mapping systems have been proposed in the past to show the catheter position in 3-D within a registered pre-operative data set . While they promise to save X-ray dose, they add effort and cost to the procedure. In addition, mapping systems are virtual reality systems. They do not allow to instantly confirm catheter positions under X-ray with respect to detailed 3-D cardiac anatomy. In some instances, they may even be off with respect to the underlying anatomy. Augmented fluoroscopy, using either CT, MRI or C-arm CT, overlaying a pre-operative data set onto live fluoroscopic images can facilitate more precise catheter navigation and also reduce fluoroscopy time. Unfortunately, catheter navigation under augmented fluoroscopy is compromised by cardiac and respiratory motion. A first approach to overcome this problem by providing a motion compensated overlay has been proposed here. It involved tracking of commonly used circumferential mapping catheters. As atrial fibrillation (Afib) therapy takes place in the vicinity of the circumferential mapping catheter, catheter tracking can be assumed to capture the motion of the relevant treatment region correctly if the device has been firmly positioned. Fortunately, we can count on the physicians to provide us with a stable wall contact, as it is in their best interest. Otherwise complete isolation of the pulmonary veins (PVs) may fail due to undetected residual PV-atrial electrical connections. The intial proposed method generates a 3-D model of the catheter and applies an unconstrained 2-D/3-D registration to register the catheter model to biplane fluoroscopy images. In the first approach, a filter-based technique using a vessel enhancement filtering was used. The method was later extended by integrating a learning-based catheter segmentation . However, this technique still required simultaneous biplane imaging. An approach for monoplane fluoroscopic imaging was introduced here considering only a 2-D catheter and moving the overlay. That is, the projection of the pre-operative 3-D data set is shifted on the live fluoroscopic images in sync with the cardiac and respiratory motion. The last method might be the approach of choice if only a monoplane C-arm system is available. In EP labs equipped with biplane C-arm systems, often only one imaging plane is used at a time to reduce the dose to the patient. In this case, the methods suggested here and here are not applicable. The 2-D method described here is not ideal either, as it requires re-initialization of the catheter model as soon as the C-arm view direction changes.
Methods
To improve the mono-plane situation, we propose a constrained 2-D/3-D registration to perform motion compensation using a 3-D catheter model. The generation of a 3-D catheter model requires only a single biplane shot. This way, the increase of X-ray radiation to the patient is kept to a minimum. In the first step of our method, a 3-D model of the circumferential mapping catheter is generated. A single biplane frame is required to manually initialize the model generation step. In the second step of our method, we segment the catheter in the fluoroscopic images. In the third step of our method, the actual motion compensation is performed by a constrained model-based 2-D/3-D registration.
Evaluation and Results
Evaluation was performed using a leave-one-out validation approach. The tracking accuracy was measured in comparison to a gold-standard segmentation. The sequence considered for testing was not included in the respective training data set. For evaluation, 13 clinical biplane sequences were available. Our data was taken from six different patients at one clinical site. They were recorded on an AXIOM Artis dBC biplane C-arm system (Siemens AG, Healthcare, Forchheim, Germany). For each sequence, a 3-D model was generated as described in Sec. 2. Afterwards, our method was evaluated by considering each imaging plane of the biplane sequences independently. This way, we obtained 26 sequences with a total frame number of 938 frames to evaluate catheter tracking. A 2-D tracking error was obtained by calculating the average 2-D distance of the projected catheter model to the goldstandard segmentation used for training. The average error, minimum error, and maximum error for each sequence are illustrated in Fig. 1. Our method yielded an overall mean error of 0.58 mm, an overall minimum error of 0.08 mm, and an overall maximum error of 1.62 mm. Since the motion compensation is performed in 3-D, a 3-D error can estimated as well. To this end, the tip of circumferential mapping catheter was manually localized in 3-D using triangulation from two views. The available 3-D trajectories of the catheter tip were taken as a gold-standard for the observed 3-D motion.
In one sequence, the 3-D error turned out rather high, because the catheter moved significantly perpendicular to the optical plane, see Fig. 2. However, in cases where the circumferential mapping catheter was firmly placed at a pulmonary vein no such movement occurred, and we were able move the overlay well in sync with the 3-D motion. This method here is still superior regarding the 3-D error, but its better accuracy comes at the cost of simultaneous biplane fluorosopy, i.e., increased X-ray dose.
Fig. 1. Two-dimensional tracking error for all 26 sequences. For each sequence, the average error, minimum error, and maximum error is given. An overall mean error of 0.58 mm, an overall minimum error of 0.08 mm, and an overall maximum error of 1.62 mm was achieved.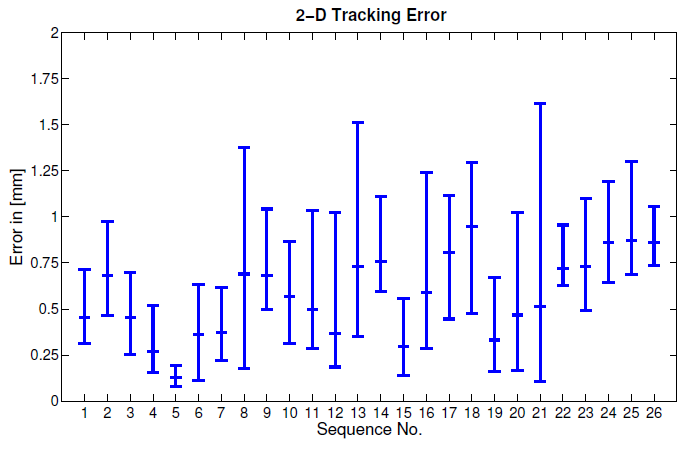 |
Fig. 2. Three-dimensional tracking error for all 26 sequences in comparison to the observed 3-D motion. For each sequence, the average, minimum, and maximum for the tracking error as well as the observed motion is given. On average, we observed an overall mean error of 2.12 mm, an overall minimum error of 0.01 mm, and an overall maximum error of 11.01 mm was achieved.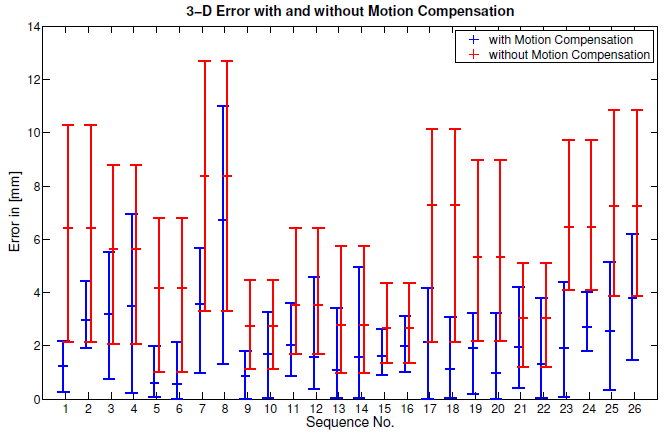 |
Discussion and Conclusions
Fig. 3. A comparison showing the difference if motion compensation is considered or not. (a) One frame of sequence 17 without motion compensation. (b) Shows the same frame of sequence 17 as in (a) but this time with motion compensation.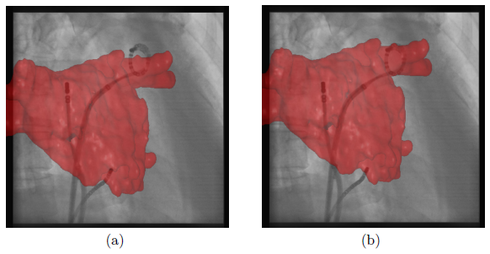 |
The 2-D tracking error of our proposed method is in the same range as 2-D reference method, see here. But instead of performing only a 2-D/2-D registration, we now rely on a constrained 2-D/3-D registration involving a 3-D catheter model. The advantage of a 3-D catheter model is that re-positioning of the C-arm is possible whereas a 2-D catheter representation needs to be re-initialized if view directions change. Nevertheless, a pure 2-D approach is the method of choice if only a monoplane fluoroscopic system is available. In addition, our new approach is learning-based whereas the approach is filter-based. Therefore, our new method requires a training phase for the segmentation of the catheter. Note, however, that by using a learning-based method we might be able to improve the tracking results. At least, this is what we observed for the biplane approach, see here. Here, the filter-based approach yielded a 2-D tracking error of 1.0 mm and a 3-D tracking error of 0.8 mm. Using a learning-based method, the errors were reduced to 0.8 mm in 2-D and to 0.7 mm in 3-D, see here.
The dedicated biplane method yields a smaller 3-D error than our new method, but it requires simultaneously biplane fluoroscopy. In our current technique, we provide only a limited 3-D motion compensation but do not require simultaneously biplane fluoroscopy as in previous work, see here. The limitation of our method is mainly related to the fact that motion along the viewing direction cannot be taken into account as depth information is hard to determine from mono-plane projection images. To achieve a depth correction, a perfect segmentation of the catheter would be required and, in addition, we would also need to know the exact dimensions of the catheter in 3-D, i.e., its diameter and its thickness. Any noise in the 2-D segmentation or at the 3-D model would decrease the accuracy of the depth estimate. To avoid this problem we have chosen to use only search directions parallel to the imaging plane. As a price to pay, we accept that the 3-D error will not be as good as in here. As in the previously presented methods, our new approach does not require a perfect segmentation of the circumferential mapping catheter, because the registration method can compensate for segmentation errors. For comparison, electro-anatomic mapping systems provide a mean tracking error of 0.7 mm which is comparable to our mean tracking error of 0.58 mm. In addition, our reference catheter, the circumferential mapping catheter, is directly at the site of ablation. Hence, it enables motion compensation for respiratory and cardiac motion without the need to separate these two motion patterns or the requirement to synchronize with ECG. Since we do not need to record the ECG signal, a stand-alone version of an augmented-reality, motion compensated fluoroscopy system is possible. A comparison between a frame with and without motion compensation is presented in Fig. 3. In a clinical setup, a physician working on a biplane system is likely to use his imaging planes in an alternating way. For such a clinical use case, our newly proposed method provides a significant advantage over the previously introduced methods, see here and here, in terms of accuracy and practicality.
Full Paper
Full paper available here.
|